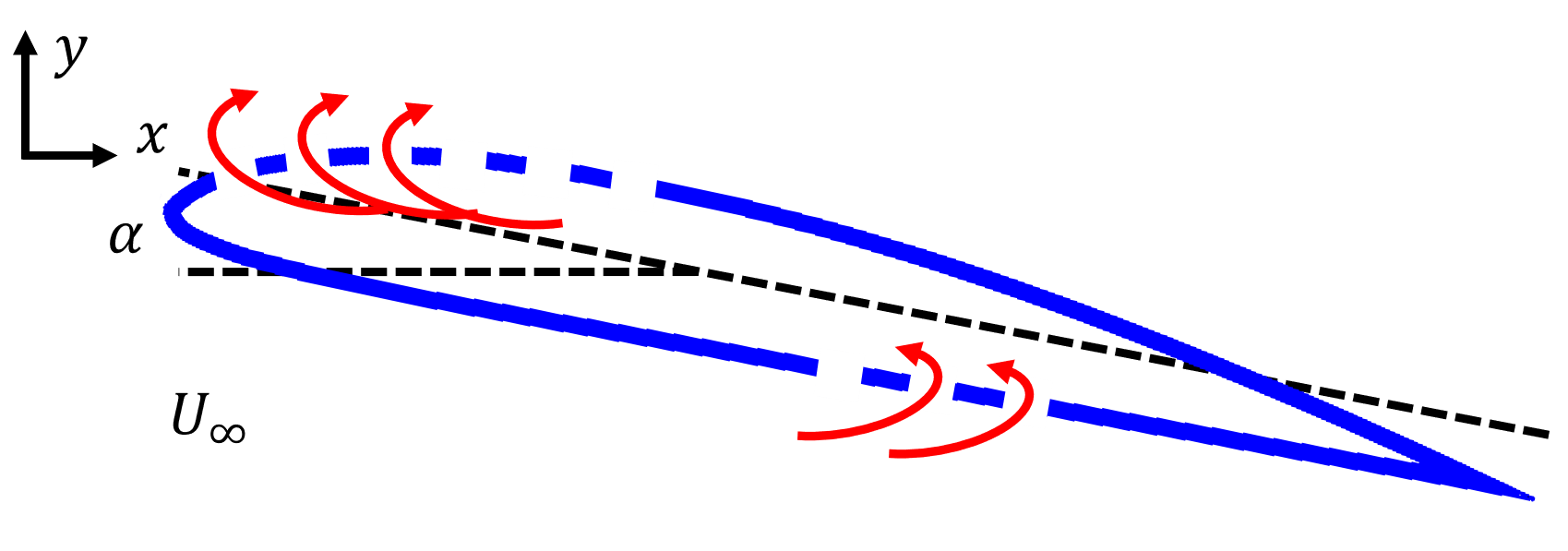
Active flow control techniques can improve aircraft maneuverability and efficiency. The experimental/theoretical research program investigates fundamental physics of controlled interactions between wing surfaces and the embedding flow to effect tunable structural and aeroelastic properties that are controlled by the aerodynamic load distributions present in flight. The interactions between the aerosurfaces and the flow, and therefore the aerodynamic loads, are regulated by active distributed air bleed that is driven through the wing’s aerodynamic surfaces by pressure differences in flight and is regulated by low-power integrated louvers (Figure 1). In using the pressure difference as the main source of flow control, we eliminate the need for complex and heavy actuators which are usually required to turn the embedding flow and allows for a lighter and more customizable wing. From the structural perspective, the controlled aerodynamic loads are exploited to effectively vary the stiffness of the wing and induce its deflections in bending and twist.

The effects of fluidic bleed actuation for variation of the aerodynamic loads on a wing were investigated using a modular semi-span wind tunnel wing model using direct force measurements and a motion analysis (Figure 2). The Clark-Y model contain bleed ports on both the pressure and suction side to drive the flow. The effect of the position of the bleed ports on the aerodynamic properties of the wing are highlighted in Figure 3a-d that show the incremental changes in the aerodynamic loads lift, drag, pitching moment and rolling moment, respectively relative to the sealed baseline. These data show that for a selected angle of attack, bleed actuation can either increase or decrease the lift and thereby the loading on the wing surface and potentially control its deformation and displacement. The bleed through different spanwise panels results in different flow effects and aerodynamic loads which is also demonstrated in the increments of the rolling moments in Figure 3d. It is also interesting to note that the present bleed configurations not only lead minimal penalty in drag (α < 10o), the variations in overall drag relative to the baseline are nearly invariant with bleed configuration.

An example of the effect of spanwise-non-uniform bleed on the flow and aerodynamic loads over the wing model is captured using stereo PIV measurements in the spanwise cross-stream (y–z) plane normal to the free stream through the wing’s near wake (c/3 downstream of its trailing edge). Figure 4a.i-a.v shows upstream views of distributions of the streamwise vorticity concentrations in the y–z plane in the baseline flow (in the absence of bleed actuation) at angles of attack α = 8°, 14°, 16°, 18°, and 20°, respectively. These data show that the changes in the aerodynamic loads effected by bleed are accompanied by the formation of a pair of counter-rotating concentrations of vorticity that might be thought of as similar to the effect of an upward-deflected flap segment. The vortex patterns are evident in the spanwise distributions of the sectional incremental lift ΔCl(z) in Figures 4c.i-c.v. These distributions of ΔCl(z) indicate the ability of the bleed to induce spanwise-compact changes in the load distribution on the wing model that is crucial from the standpoint of spatial modifications of the apparent structural characteristics of the wing. Further insight into the flow in this domain is provided by considering the spanwise distribution of streamwise deficit of streamwise momentum in the wake relative to the free stream ΔPx(z) in Figures 4d.i-d.v. These data show that, while the bleed results in an increase in the near wake’s momentum deficit downstream from the bleed ports position, it leads to a concurrent decrease in the deficit in the root domain indicating that the streamwise vorticity induced by the bleed results flow attachment and reduction in sectional drag.

Supported by the Air Force Office of Scientific Research (AFOSR)